Cancer du sein
Le cancer du sein est le type de cancer spécifique le plus répandu chez les femmes dans le monde (Sung et. al., 2021). Chez les femmes, le cancer du sein représente 1 cas de cancer sur 4 et 1 décès par cancer sur 6, se classant au premier rang en termes d'incidence dans la grande majorité des pays (159 pays sur 185) et de mortalité dans 110 pays (Sung et. al., 2021). La plupart des cas surviennent chez les femmes âgées de plus de 50 ans, mais ils peuvent également toucher des femmes plus jeunes. D'autres facteurs de risque comprennent une prédisposition génétique, des antécédents familiaux, l'apparition précoce des règles, un traitement hormonal substitutif, la consommation d'alcool et l'obésité (Łukasiewicz et al., 2021).
Le sein est composé de lobules producteurs de lait, d'un système de canaux de transport et de tissu adipeux (Bazira et al., 2021). Tous les cancers du sein proviennent des cellules tapissant les unités lobulaires des canaux terminaux (l'unité fonctionnelle du sein) des canaux collecteurs. Le type de cancer du sein masculin le plus courant est le carcinome canalaire invasif, qui prend naissance dans les canaux galactophores et envahit les tissus voisins (Harbeck et al., 2019). Le développement du cancer du sein implique des mutations génétiques qui provoquent une prolifération cellulaire incontrôlée ainsi que les gènes BRCA1 et BRCA2, impliqués dans la réparation de l'ADN (Harbeck et al., 2019). Les récepteurs des oestrogènes et de la progestérone jouent un rôle important dans la physiopathologie ; tous les patients atteints de tumeurs exprimant ces récepteurs devraient recevoir un traitement hormonal pour bloquer l'activité des récepteurs des oestrogènes (Harbeck et al., 2019).
Le cancer du sein peut se manifester de différentes manières. Les caractéristiques cliniques les plus courantes sont la présence d’une masse dans le sein, des modifications de la taille du mamelon, un écoulement du mamelon et des modifications cutanées ainsi qu'une infection et/ou une inflammation du sein (Koo et al., 2017). Le cancer du sein à un stade précoce est souvent asymptomatique, ce qui souligne l'importance du dépistage systématique (Kalager et al., 2010).
Le cancer du sein est généralement diagnostiqué lors d’un dépistage ou en présence d’un symptôme (douleur ou masse palpable) qui déclenche un examen diagnostique (McDonald et al., 2016). Celui-ci est complété par des techniques d’imagerie permettant de rechercher des anomalies et de les caractériser plus en détail (McDonald et al., 2016). Une biopsie mammaire est généralement réalisée pour confirmer la présence d'un cancer en cas de suspicion, et permet également de déterminer son type spécifique si la lésion est cancéreuse (McDonald et al., 2016). Le cancer du sein est classé en fonction de l'étendue de la tumeur, de la propagation aux ganglions lymphatiques voisins, de la propagation à des sites distants, du statut des récepteurs aux oestrogènes, du statut des récepteurs de la progestérone, du statut HER2 et du grade du cancer (McDonald et al., 2016).
Il existe différents types de cancer du sein, et le traitement peut varier en fonction des caractéristiques moléculaires de la maladie, du stade, du type de cancer et du statut des récepteurs du patient (Hong et Xu, 2022). Le traitement implique généralement une association de différentes modalités et une équipe multidisciplinaire de professionnels de la santé (Hong et Xu, 2022). Les options chirurgicales vont des procédures conservatrices du sein à la mastectomie, où le sein entier est retiré (Hong et Xu, 2022). L'ablation des ganglions lymphatiques peut également s’avérer nécessaire pour évaluer l'étendue de la propagation du cancer (Hong et Xu, 2022). La radiothérapie est souvent utilisée après un traitement conservateur du sein ou une mastectomie (avec facteurs de risque) (Hong et Xu, 2022). La chimiothérapie systémique peut être administrée avant ou après la chirurgie, selon la situation spécifique (Hong et Xu, 2022). Les cancers du sein à récepteurs hormonaux positifs peuvent être traités par des médicaments qui bloquent les effets des oestrogènes et de la progestérone. L'immunothérapie est une option thérapeutique émergente pour certains cancers du sein, aidant le système immunitaire à reconnaître et à attaquer les cellules cancéreuses (Hong et Xu, 2022).
Techniques d'imagerie
Mammographie numérique
La mammographie numérique est la technique la plus souvent utilisée pour le dépistage du cancer du sein. Il s'agit d'une technique de sommation bidimensionnelle dans un tube à rayons X émet des rayons X, qui sont absorbés à différents degrés par les tissus et mesurés par un détecteur situé à l'autre extrémité. Les tissus plus denses apparaissent plus clairs sur les images résultantes que les tissus moins denses. Les seins sont comprimés pendant l'acquisition de l'image pour étendre le tissu mammaire sur une plus grande surface (Ikeda, 2011a). Cette technique permet de réduire le chevauchement entre les différents composants du tissu mammaire, de diminuer la diffusion des rayons X qui passent et d’améliorer le contraste. Deux vues de chaque sein sont généralement acquises : crânio-caudale (CC) et médio-latérale (MLO) (Ikeda, 2011a).
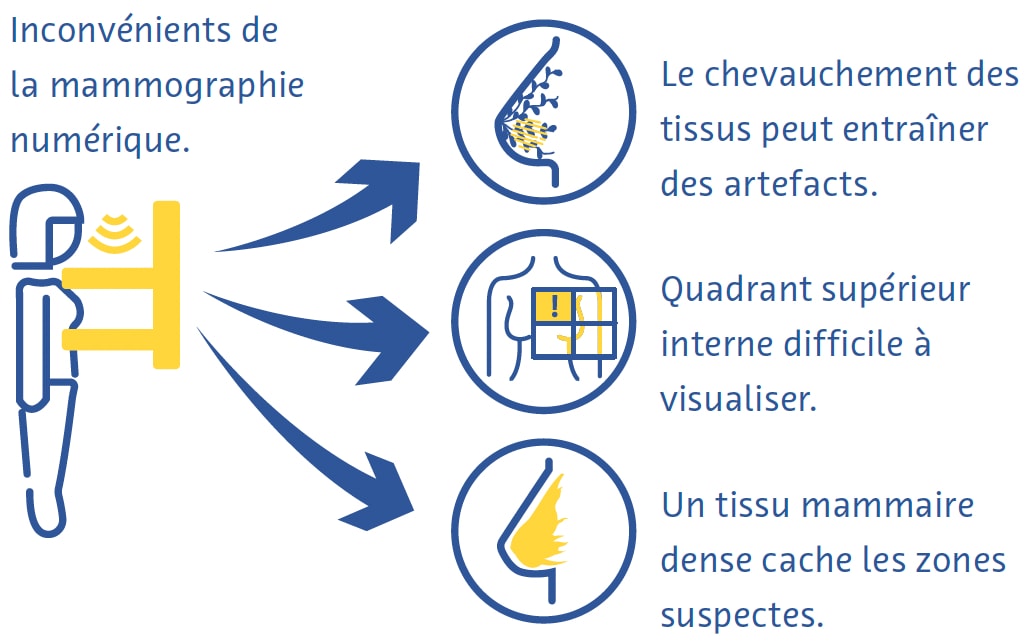
La mammographie numérique est une technique rapide et utile pour le dépistage du cancer du sein, mais elle présente néanmoins des inconvénients (Ikeda, 2011a). La compression mammaire peut être douloureuse et, malgré la compression, le chevauchement de différents tissus conduit souvent à des artefacts (Ikeda, 2011a). Le quadrant supérieur interne du sein, moins mobile car fixé à la paroi thoracique, est particulièrement difficile à visualiser en mammographie (Ikeda, 2011a). Le cancer peut également être très difficile à détecter à la mammographie dans les seins comportant une forte proportion de tissus denses (Ikeda, 2011a).
Tomosynthèse mammaire numérique
La tomosynthèse mammaire numérique (TMN) implique l'acquisition d'images à l'aide d'une source de rayons X qui se déplace le long d'un arc d'excursion. De fines tranches sont reconstruites, permettant des capacités d'imagerie 3D destinées à limiter autant que possible l'influence du tissu mammaire qui se chevauche. Cette méthode est particulièrement utile pour l'imagerie des lésions mammaires situées dans un parenchyme mammaire hétérogène et dense. Une étude a révélé que la TMN est plus sensible pour la détection du cancer du sein que la mammographie numérique (MN). La TMN peut être combinée avec la MN et une étude a révélé que l'utilisation d'une combinaison de ces techniques améliore la détection du cancer du sein (Alabousi et al., 2020 ; Lei et al., 2014 ; Skaane et al., 2019) et peut être combinée avec la mammographie. Cependant, la TMN prend plus de temps à acquérir que la mammographie et souffre d’artefacts de mouvements et autres (Tirada et al., 2019).
Échographie
Dans l’échographie diagnostique, un transducteur émet des ondes sonores à haute fréquence qui traversent les tissus, rebondissent sur eux et créent des « échos » qui sont réfléchis et détectés par le transducteur. Ces échos sont ensuite traités pour créer des images en temps réel sur un moniteur en fonction du temps nécessaire aux échos pour se déplacer vers les tissus et revenir. Il s’agit d’une technique sûre et relativement peu coûteuse qui est souvent utilisée en complément de la mammographie (Ikeda, 2011b), notamment pour évaluer plus en détail un résultat palpable ou mammographique.
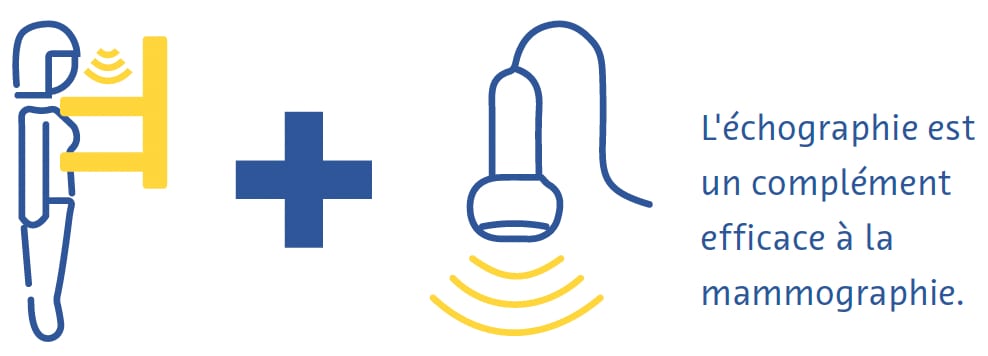
Elle peut même être utilisée comme modalité de dépistage primaire chez les femmes de moins de 30 ans ou chez les femmes enceintes ou allaitantes (Dixon, 2008 ; Ikeda, 2011b). L'échographie est très utile pour déterminer si une masse est kystique ou solide, quels types de marges elle présente et sa vascularisation (Dixon, 2008 ; Ikeda, 2011b). Elle permet également de détecter d'autres masses et ganglions lymphatiques axillaires suspects (Dixon, 2008 ; Ikeda, 2011b). Son principal inconvénient est que la qualité de l’examen dépend fortement de l’opérateur (Dixon, 2008 ; Ikeda, 2011b).
Imagerie par résonance magnétique
À l’aide d’un puissant champ magnétique et d’une série d’ondes radiofréquences, l’imagerie par résonance magnétique (IRM) perturbe les noyaux d’hydrogène dans les tissus pour créer des images transversales détaillées du corps (Daniel et Ikeda, 2011 ; Mann et al., 2019). Étant donné que les tissus de compositions différentes réagissent de différentes manières à cette perturbation, l’IRM peut très bien détecter même des différences subtiles entre les types de tissus mous et est considérée comme la modalité la plus sensible pour diagnostiquer le cancer du sein (Daniel et Ikeda, 2011 ; Mann et al., 2019). Elle est principalement utilisée pour dépister les patients à haut risque selon les facteurs de risque génétiques ou acquis (Daniel et Ikeda, 2011).
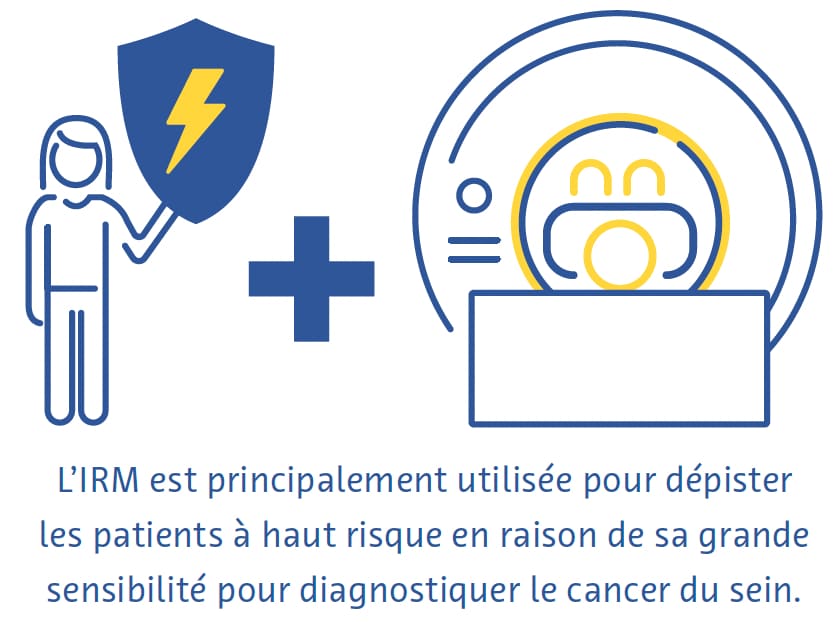
L’IRM mammaire nécessite des antennes mammaires dédiées qui transmettent les ondes radiofréquences et reçoivent le signal généré. Les images sont souvent acquises avec une résolution spatiale dans le plan de 1 mm, une épaisseur de coupe inférieure à 3 mm et une suppression du signal provenant des lipides. Les séquences couramment utilisées comprennent les images pondérées en T2, l'imagerie pondérée en diffusion et l'IRM dynamique avec contraste amélioré. Pour réduire les faux positifs dus à des modifications non spécifiques du parenchyme mammaire, il est préférable de l'effectuer entre le 7e et le 13e jour du cycle menstruel (Daniel et Ikeda, 2011). Contrairement à la mammographie, l'IRM n'utilise pas de rayonnements ionisants et produit des images tridimensionnelles qui facilitent la détection de très petites lésions (DeMartini et Lehman, 2008 ; Shahid et al., 2016). L'IRM permet également une évaluation plus détaillée de la paroi thoracique que la mammographie et l'échographie (DeMartini et Lehman, 2008). Les inconvénients de l'IRM mammaire comprennent une faible sensibilité aux micro-calcifications, un coût élevé et le fait qu'elle soit contre-indiquée chez les personnes portant certains implants métalliques (Daniel et Ikeda, 2011).
Défis du dépistage et du diagnostic
Malgré les preuves du bénéfice global du dépistage du cancer du sein (Dibden et al., 2020 ; Kalager et al., 2010 ; Tabár et al., 2019), ce dernier souffre de plusieurs défis techniques et logistiques. Plus de la moitié des femmes dépistées chaque année pendant 10 ans auront un test faussement positif (Hubbard et al., 2011). Cela a des conséquences vastes et significatives, notamment le fardeau physique et émotionnel des biopsies inutiles et l'augmentation des coûts des soins de santé (Nelson, Pappas et al., 2016 ; Ong et Mandl, 2015). Le cancer du sein passe souvent inaperçu lors du dépistage, en particulier chez les femmes aux seins denses (Banks et al., 2006).
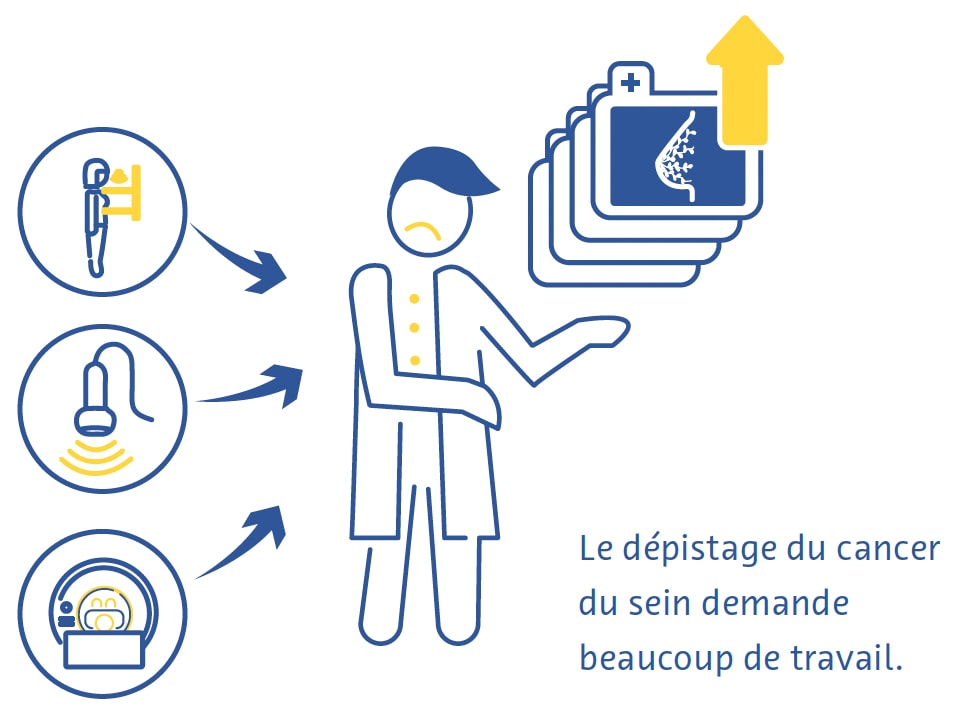
Le dépistage du cancer du sein nécessite du personnel hautement qualifié, notamment des radiologues et des radiographes, dont il existe actuellement une pénurie mondiale (Moran et Warren-Forward, 2012 ; Rimmer, 2017 ; Wing et Langelier, 2009). Ce problème est aggravé par le fait que la norme de soins en matière de dépistage du cancer du sein dans de nombreux pays européens est que chaque examen est lu indépendamment par deux radiologues (Giordano et al., 2012) et que, dans certains pays, comme les États-Unis, les obstacles à l'obtention de l'autorisation d'interpréter des mammographies sont élevés en raison des normes strictes de certification professionnelle (Food and Drug Administration, 2001).
Il existe également des obstacles importants à l’adoption du dépistage du cancer du sein dans le monde. Ceux-ci incluent le manque ou l’accès difficile aux programmes de dépistage, le manque de connaissances ou l’incompréhension des avantages de ces programmes, ainsi que les obstacles sociaux et culturels (Mascara et Constantinou, 2021).
Rôle de l'intelligence artificielle
Améliorations techniques
Jusqu’à présent, peu d’études publiées concernaient directement l’utilisation de l’IA pour apporter des améliorations techniques aux examens des seins. Une application disponible dans le commerce fournit aux radiologues un retour d'information en temps réel sur l'adéquation du positionnement du patient sur les mammographies. (Volpara Health, 2022). D'autres applications de l'IA se sont concentrées sur la réduction des doses de rayonnement (J. Liu et al., 2018), l'amélioration de la reconstruction des images (Kim et al., 2016) et la réduction du bruit et des artefacts sur la TMN (Garrett et al., 2018).
La TMN est fréquemment associée à la mammographie numérique pour le dépistage du cancer du sein, qui double la dose de rayonnement reçue par les patients (Svahn et al., 2015). Pour éviter cela, la génération de mammographies synthétiques à partir de données issues de la TMN suscite un intérêt croissant (Chikarmane et al., 2023). Dans une vaste étude prospective norvégienne, les précisions de la TMN combinée à la mammographie numérique ou à la mammographie synthétique pour la détection du cancer du sein étaient très similaires (Skaane et al., 2019). Des études récentes ont cherché à améliorer la qualité de la mammographie synthétique grâce à l’IA, avec des résultats prometteurs (Balleyguier et al., 2017 ; James et al., 2018).
Améliorations du diagnostic
Évaluation de la densité mammaire
Le tissu mammaire dense visible à la mammographie représente le tissu fibro-glandulaire. Les femmes ayant des seins denses présentent un risque de cancer du sein 2 à 4 fois plus élevé que les femmes dont les seins contiennent plus de tissu mammaire gras (Byrne et al., 1995 ; Duffy et al., 2018 ; Torres-Mejía et al., 2005). De plus, la sensibilité de la mammographie pour le cancer du sein est 20 à 30 % plus faible dans les seins denses que dans les seins moins denses (Lynge et al., 2019). La norme de soins en matière d'évaluation de la densité mammaire utilise la classification BI-RADS (Berg et al., 2000).
Plusieurs grandes études ont étudié le potentiel de l’évaluation automatique de la densité mammaire sur les mammographies à l’aide d’outils basés sur l’IA. Un réseau neuronal convolutif (RNC) formé sur 14 000 mammographies et testé sur près de 2 000 mammographies a classé la densité mammaire en « densité dispersée » ou « densité hétérogène » avec une aire sous la courbe (ASC) de 0,93 (Mohamed et al. , 2018). Une autre étude a utilisé un RNC capable de classification BI-RADS binaire et quadri-directionnelle et s'est entraîné sur plus de 40 000 mammographies (Lehman et al., 2019). Dans un ensemble de données de test de plus de 8 000 mammographies, l’étude a trouvé un bon accord sur la densité mammaire entre l’algorithme et les radiologues individuels (kappa = 0,67) ainsi que le consensus de cinq radiologues (kappa = 0,78) (Lehman et al., 2019).
Détection du cancer du sein
Dans une revue systématique incluant 82 études utilisant l'IA pour la détection du cancer du sein avec diverses normes de référence, les auteurs ont trouvé une ASC de 0,87 pour les études utilisant la mammographie, de 0,91 avec l'échographie, de 0,91 avec la TMN et de 0,87 avec l'IRM (Aggarwal et al., 2021). Ces résultats sont prometteurs, mais des comparaisons directes entre les algorithmes basés sur l’IA et les radiologues révèlent des possibilités d’amélioration. Dans une autre revue systématique d'études utilisant soit l'histopathologie, soit le suivi (pour les femmes au dépistage négatif) comme référence, 94 % des 36 RNC identifiés étaient moins précis qu'un seul radiologue, et tous étaient moins précis que le consensus de 2 radiologues ou plus lorsqu’ils sont utilisés comme système autonome (Freeman et al., 2021). Les données actuelles ne soutiennent donc pas l’utilisation de l’IA comme stratégie autonome de détection du cancer du sein.
Prédiction du cancer du sein
L’IA s’est révélée prometteuse pour prédire le risque de développer un cancer du sein sur la base de mammographies de dépistage, soit en fournissant une meilleure évaluation de la densité mammaire, un facteur de risque établi pour le cancer du sein (Duffy et al., 2018), soit en détectant des caractéristiques d’imagerie subtiles qui sont précurseurs du cancer (Batchu et al., 2021). Plusieurs études ont utilisé des modèles basés sur l’IA pour prédire le risque de développer un cancer du sein à l’avenir sur la base de mammographies (Batchu et al., 2021 ; Geras et al., 2019).
Un RNC formé sur près de 1 000 000 images mammographiques a montré une ASC de 0,65 pour la prévision du développement futur du cancer du sein, contre 0,57 à 0,60 pour les scores de densité mammaire obtenus à partir d’une mammographie conventionnelle (Dembrower, Liu et al., 2020). Une étude plus petite a révélé une ASC de 0,73 pour une méthode basée sur un RNC pour prédire le cancer du sein à partir d'images mammographiques normales. (Arefan et al., 2020). Un autre algorithme d'apprentissage profond a montré une ASC de 0,82 pour prédire les cancers d'intervalle (cancers détectés dans les 12 mois suivant une mammographie négative) par rapport à 0,65 pour l'évaluation visuelle BI-RADS de la densité mammaire (Hinton et al., 2019). Un autre modèle basé sur l'apprentissage profond qui incorporait à la fois les facteurs de risque et les résultats mammographiques pour prédire le risque de cancer du sein présentait une ASC allant jusqu'à 0,7, dépassant la précision des modèles prédictifs basés uniquement sur les facteurs de risque ou les résultats mammographiques. (Yala, Lehman et al., 2019).
Améliorations de l'efficacité
Le grand nombre d’examens mammographiques et la pénurie de radiologues qualifiés ont fait de l’amélioration de l’efficacité l’un des domaines de recherche les plus intéressants sur l’utilisation de l’IA dans le cancer du sein.
Dans une étude, les auteurs ont simulé un flux de travail dans lequel les mammographies étaient interprétées par un radiologue et un modèle d'apprentissage profond, la décision étant considérée comme définitive en cas d’accord (McKinney et al., 2020). Un deuxième radiologue n'était consulté qu'en cas de désaccord, ce qui était associé à une réduction de 88 % de la charge de travail du deuxième radiologue avec une valeur prédictive négative de plus de 99,9 % (McKinney et al., 2020).
Dans le cadre d’un premier essai clinique randomisé de grande envergure réalisé en Suède, environ 80 000 femmes ont été randomisées pour faire pré-lire ou non leurs mammographies de dépistage par un RNC (Lång et al., 2023). Dans le groupe d'intervention, seules les mammographies ayant reçu un score de probabilité élevée de malignité ont bénéficié d’une deuxième lecture (les autres ont été lues par un seul radiologue) et les résultats ont été comparés à une double lecture conventionnelle sans l'aide de l'algorithme. Dans une analyse intermédiaire des données provenant des 80 000 femmes, les deux groupes de l’étude ont montré un taux de faux positifs identique de 1,5 %. La valeur prédictive positive du rappel était de 28,3 % dans le groupe d'intervention et de 24,8 % dans le groupe témoin, et la stratégie a réduit la charge de travail de 44,3 % (Lång et al., 2023).
D’autres études ont utilisé l’IA pour présélectionner les mammographies, en triant celles présentant un faible risque de cancer et en montrant uniquement celles présentant un risque élevé de cancer à un radiologue. Une étude américaine a utilisé un flux de travail simulé impliquant un RNC formé sur plus de 212 000 mammographies et testé sur plus de 26 000 à cette fin (Yala, Schuster et al., 2019). Le flux de travail utilisant l'algorithme avait une sensibilité non inférieure au cancer du sein (90,1 % contre 90,6 %) et une spécificité légèrement supérieure à celle des radiologues travaillant seuls (94,2 % contre 93,5 %) et était associé à une charge de travail inférieure de 19,3 % (Yala, Schuster et coll., 2019). Une étude plus petite menée en Espagne a révélé une diminution de 72,5 % de la charge de travail en utilisant l'IA pour trier uniquement les cas de TMN à haut risque pour une deuxième lecture par un radiologue et de 29,7 % en utilisant l'IA pour envoyer uniquement les études de TMN à haut risque pour lecture par un deuxième radiologue, par rapport au flux de travail de mammographie traditionnels à la double lecture (Raya-Povedano et al., 2021). L’étude a également constaté une sensibilité non inférieure de cette stratégie consistant à utiliser l’IA pour trier les cas de mammographie et de TMN à haut risque pour une seconde lecture par rapport aux flux de travail standard de mammographie à double lecture et de TMN (Raya-Povedano et al., 2021). Dans une étude suédoise, une stratégie similaire utilisant un algorithme d'IA disponible dans le commerce a donné un taux de faux négatifs ne dépassant pas 4 % et l'algorithme a démontré la capacité de détecter 71 cancers potentiels supplémentaires pour 1 000 examens, plus qu'une double lecture négative par radiologues chez les patients jugés à risque très élevé par l'algorithme d'IA (Dembrower, Wåhlin, et al., 2020).
Dans une étude portant sur plus d'un million de mammographies réalisées sur huit sites de dépistage et trois fabricants de dispositifs, un algorithme d'apprentissage profond disponible dans le commerce a trié 63 % des cas sans autre examen, sur la base d'évaluations de haute confiance des examens (Leibig et al., 2022). Les autres examens, pour lesquels la confiance dans l'algorithme était faible, ont été présentés aux radiologues. Cette stratégie a amélioré la sensibilité des radiologues (par rapport à la lecture spontanée) de 2,6 à 4 % et la spécificité de 0,5 à 1,0 % (Leibig et al., 2022).
Défis et orientations futures
Plusieurs défis éthiques, techniques et méthodologiques associés à l’utilisation de l’IA dans le dépistage du cancer du sein fournissent un cadre pour orienter les recherches futures sur ce sujet (Hickman et al., 2021).
La plupart des outils basés sur l'IA se sont jusqu'à présent concentrés sur la mammographie numérique (Aggarwal et al., 2021), mais d'autres techniques d'examen telles que la TMN et l'IRM présentent des avantages uniques (Alsheik et al., 2019 ; Mann et al., 2019) et sont susceptibles de jouer un rôle plus important dans le dépistage du cancer du sein à l’avenir. Cependant, étant donné que la TMN et l’IRM sont des techniques tomographiques produisant des résultats tridimensionnels, leur traitement à l’aide d’outils basés sur l’IA nécessitera plus d’espace de stockage et de puissance de calcul (Prevedello et al., 2019).
L'incidence, la présentation et l'issue du cancer du sein sont liées à plusieurs facteurs sociodémographiques, notamment la race et l'origine ethnique (Hirko et al., 2022 ; Hu et al., 2019 ; Martini et al., 2022). La formation d’outils basés sur l’IA sur des ensembles de données représentant une population diversifiée est essentielle pour garantir la généralisation et permettre qu’ils soient bénéfiques au plus grand nombre de personnes possible.
Les performances globales de l’IA pour la détection du cancer du sein étaient impressionnantes. Cependant, il convient de noter qu’une sensibilité non inférieure de l’IA à celle des radiologues pour détecter le cancer du sein n’a pu être prouvée dans une étude (Lauritzen et al., 2022). De plus, la qualité des preuves derrière de nombreuses études sur ce sujet est préoccupante. Une revue systématique portant sur l’exactitude des outils basés sur l’IA pour la détection du cancer du sein a identifié plusieurs domaines d’amélioration potentiels (Freeman et al., 2021). La revue n'a trouvé aucune étude prospective et les études identifiées étaient de mauvaise qualité méthodologique.
En particulier, les auteurs ont observé que des études plus petites ont montré des résultats plus positifs qui n'ont pas été reproduits dans les études plus vastes. Dans une autre revue systématique, seulement environ une étude sur dix a utilisé un ensemble de données externes pour la validation, aucune étude n'a fourni un calcul de taille d'échantillon prédéfini et de graves problèmes de biais de sélection et de normes de référence inappropriées ont été identifiés (Aggarwal et al., 2021). Ces problèmes méthodologiques pourraient potentiellement être atténués à l’avenir grâce à l’introduction de grands référentiels de données ouverts (Nguyen et al., 2023) et au respect accru des lignes directrices pour la conduite de recherches médicales basées sur l’IA (Lekadir et al., 2021 ; X. Liu et al., 2020).
Conclusion
L’intégration de l’intelligence artificielle dans les programmes de dépistage du cancer du sein est prometteuse pour optimiser la qualité des images, améliorer l’efficacité et prédire le risque futur de cancer du sein. Pour détecter le cancer du sein lors des examens de dépistage, les preuves suggèrent que l’intelligence artificielle fonctionne mieux lorsqu’elle travaille en synergie avec les radiologues. La recherche en cours est essentielle pour relever les défis associés à l’utilisation de l’IA dans le dépistage du cancer du sein, notamment en élargissant ses applications au-delà de la mammographie et en garantissant son utilisation éthique et responsable. Avec l’évolution continue des applications de l’IA, l’avenir du dépistage du cancer du sein recèle un immense potentiel en termes d’accessibilité accrue, d’intervention précoce et, à terme, d’amélioration des résultats pour les patients.
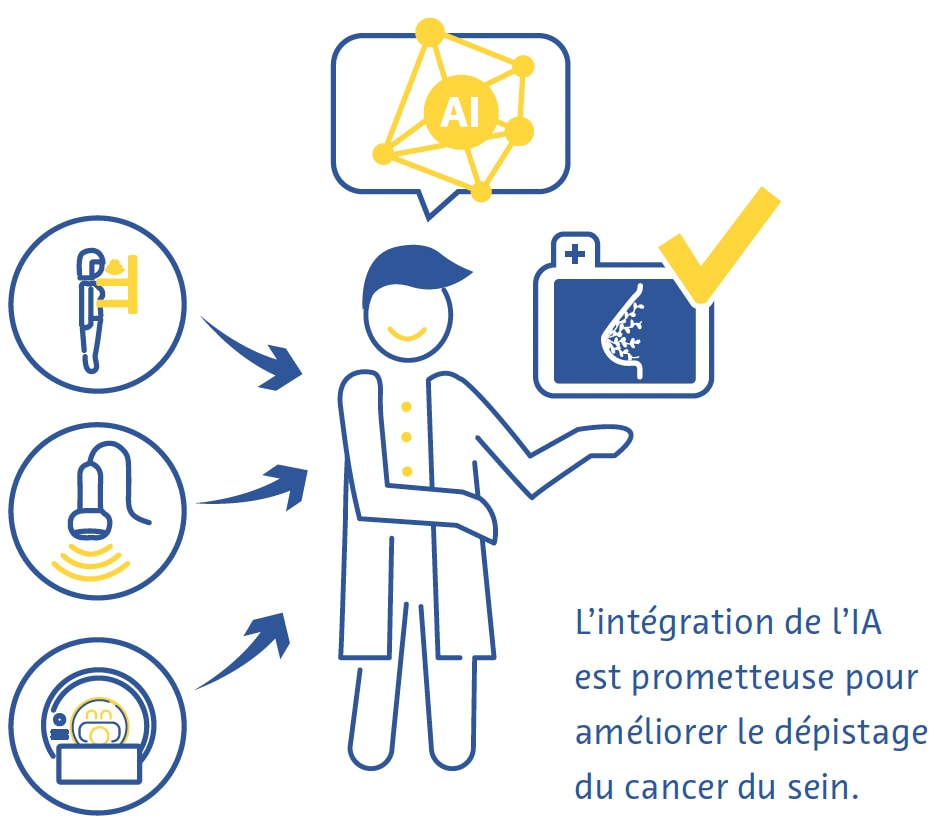
Références
Aggarwal, R., Sounderajah, V., Martin, G., Ting, D. S. W., Karthikesalingam, A., King, D., Ashrafian, H., & Darzi, A. (2021). Diagnostic accuracy of deep learning in medical imaging: a systematic review and meta-analysis. NPJ Digital Medicine, 4(1), 65.
Alabousi, M., Zha, N., Salameh, J.-P., Samoilov, L., Sharifabadi, A. D., Pozdnyakov, A., Sadeghirad, B., Freitas, V., McInnes, M. D. F., & Alabousi, A. (2020). Digital breast tomosynthesis for breast cancer detection: a diagnostic test accuracy systematic review and meta-analysis. European Radiology, 30(4), 2058–2071.
Alkabban FM and Ferguson T. (2022). Breast Cancer, 2022. In: StatPearls [Internet]. Treasure Island (FL): StatPearls Publishing.
Alsheik, N. H., Dabbous, F., Pohlman, S. K., Troeger, K. M., Gliklich, R. E., Donadio, G. M., Su, Z., Menon, V., & Conant, E. F. (2019). Comparison of Resource Utilization and Clinical Outcomes Following Screening with Digital Breast Tomosynthesis Versus Digital Mammography: Findings From a Learning Health System. Academic Radiology, 26(5), 597–605.
Arefan, D., Mohamed, A. A., Berg, W. A., Zuley, M. L., Sumkin, J. H., & Wu, S. (2020). Deep learning modeling using normal mammograms for predicting breast cancer risk. Medical Physics, 47(1), 110–118.
Balleyguier, C., Arfi-Rouche, J., Levy, L., Toubiana, P. R., Cohen-Scali, F., Toledano, A. Y., & Boyer, B. (2017). Improving digital breast tomosynthesis reading time: A pilot multi-reader, multi-case study using concurrent Computer-Aided Detection (CAD). European Journal of Radiology, 97, 83–89.
Banks, E., Reeves, G., Beral, V., Bull, D., Crossley, B., Simmonds, M., Hilton, E., Bailey, S., Barrett, N., Briers, P., English, R., Jackson, A., Kutt, E., Lavelle, J., Rockall, L., Wallis, M. G., Wilson, M., & Patnick, J. (2006). Hormone replacement therapy and false positive recall in the Million Women Study: patterns of use, hormonal constituents and consistency of effect. Breast Cancer Research: BCR, 8(1), R8.
Batchu, S., Liu, F., Amireh, A., Waller, J., & Umair, M. (2021). A Review of Applications of Machine Learning in Mammography and Future Challenges. Oncology, 99(8), 483–490. https://doi.org/10.1159/000515698
Bazira, P. J., Ellis, H., & Mahadevan, V. (2022). Anatomy and physiology of the breast. Surgery, 40(2), 79–83. https://doi.org/10.1016/j.mpsur.2021.11.015
Berg, W. A., Campassi, C., Langenberg, P., & Sexton, M. J. (2000). Breast Imaging Reporting and Data System: interand intraobserver variability in feature analysis and final assessment. AJR. American Journal of Roentgenology, 174(6), 1769–1777.
Boyd, N. F., Guo, H., Martin, L. J., Sun, L., Stone, J., Fishell, E., Jong, R. A., Hislop, G., Chiarelli, A., Minkin, S., & Yaffe, M. J. (2007). Mammographic density and the risk and detection of breast cancer. The New England Journal of Medicine, 356(3), 227–236.
Bray, F., Ferlay, J., Soerjomataram, I., Siegel, R. L., Torre, L. A., & Jemal, A. (2018). Global cancer statistics 2018: GLOBOCAN estimates of incidence and mortality worldwide for 36 cancers in 185 countries. CA: A Cancer Journal for Clinicians, 68(6), 394–424.
Breast Cancer Statistics. (2020, October 27). Susan G. Komen®. https://www.komen.org/breast-cancer/facts-statistics/breastcancer- statistics/
Byrne, C., Schairer, C., Wolfe, J., Parekh, N., Salane, M., Brinton, L. A., Hoover, R., & Haile, R. (1995). Mammographic features and breast cancer risk: effects with time, age, and menopause status. Journal of the National Cancer Institute, 87(21), 1622–1629.
Chikarmane, S. A., Offit, L. R., & Giess, C. S. (2023). Synthetic Mammography: Benefits, Drawbacks, and Pitfalls. Radiographics: A Review Publication of the Radiological Society of North America, Inc, 43(10), e230018. https://doi.org/10.1148/rg.230018
Daniel, B. L., & Ikeda, D. M. (2011). Chapter 7 - Magnetic Resonance Imaging of Breast Cancer and MRI-Guided Breast Biopsy. In D. M. Ikeda (Ed.), Breast Imaging (Second Edition) (pp. 239–296). Mosby.
DeMartini W & Lehman C, (2008). Top Magn Reson Imaging, Jun;19(3):143-50.
Dembrower, K., Liu, Y., Azizpour, H., Eklund, M., Smith, K., Lindholm, P., & Strand, F. (2020). Comparison of a Deep Learning Risk Score and Standard Mammographic Density Score for Breast Cancer Risk Prediction. Radiology, 294(2), 265–272.
Dembrower, K., Wåhlin, E., Liu, Y., Salim, M., Smith, K., Lindholm, P., Eklund, M., & Strand, F. (2020). Effect of artificial intelligence-based triaging of breast cancer screening mammograms on cancer detection and radiologist workload: a retrospective simulation study. The Lancet. Digital Health, 2(9), e468–e474.
Dibden, A., Offman, J., Duffy, S. W., & Gabe, R. (2020). Worldwide Review and Meta-Analysis of Cohort Studies Measuring the Effect of Mammography Screening Programmes on Incidence-Based Breast Cancer Mortality. Cancers, 12(4). https://doi.org/10.3390/cancers12040976
Duffy, S. W., Morrish, O. W. E., Allgood, P. C., Black, R., Gillan, M. G. C., Willsher, P., Cooke, J., Duncan, K. A., Michell, M. J., Dobson, H. M., Maroni, R., Lim, Y. Y., Purushothaman, H. N., Suaris, T., Astley, S. M., Young, K. C., Tucker, L., & Gilbert, F. J. (2018). Mammographic density and breast cancer risk in breast screening assessment cases and women with a family history of breast cancer. European Journal of Cancer, 88, 48–56.
Food and Drug Administration. (2001). The Mammography Quality Standards Act Final Regulations: Preparing for MQSA Inspections; Final Guidance for Industry and FDA.
Freeman, K., Geppert, J., Stinton, C., Todkill, D., Johnson, S., Clarke, A., & Taylor-Phillips, S. (2021). Use of artificial intelligence for image analysis in breast cancer screening programmes: systematic review of test accuracy. BMJ, 374, n1872.
Freer, P. E. (2015). Mammographic breast density: impact on breast cancer risk and implications for screening. Radiographics: A Review Publication of the Radiological Society of North America, Inc, 35(2), 302–315.
Garrett, J. W., Li, Y., Li, K., & Chen, G.-H. (2018). Reduced anatomical clutter in digital breast tomosynthesis with statistical iterative reconstruction. Medical Physics, 45(5), 2009–2022.
Geras, K. J., Mann, R. M., & Moy, L. (2019). Artificial Intelligence for Mammography and Digital Breast Tomosynthesis: Current Concepts and Future Perspectives. Radiology, 293(2), 246–259. https://doi.org/10.1148/radiol.2019182627
Giordano, L., von Karsa, L., Tomatis, M., Majek, O., de Wolf, C., Lancucki, L., Hofvind, S., Nyström, L., Segnan, N., Ponti, A., Eunice Working Group, Van Hal, G., Martens, P., Májek, O., Danes, J., von Euler-Chelpin, M., Aasmaa, A., Anttila, A., Becker, N., … Suonio, E. (2012). Mammographic screening programmes in Europe: organization, coverage and participation. Journal of Medical Screening, 19 Suppl 1, 72–82.
Harbeck, N., Penault-Llorca, F., Cortes, J., Gnant, M., Houssami, N., Poortmans, P., Ruddy, K., Tsang, J., & Cardoso, F. (2019). Breast cancer. Nature Reviews. Disease Primers, 5(1), 66. https://doi.org/10.1038/s41572-019-0111-2
Hickman, S. E., Baxter, G. C., & Gilbert, F. J. (2021). Adoption of artificial intelligence in breast imaging: evaluation, ethical constraints and limitations. British Journal of Cancer, 125(1), 15–22.
Hinton, B., Ma, L., Mahmoudzadeh, A. P., Malkov, S., Fan, B., Greenwood, H., Joe, B., Lee, V., Kerlikowske, K., & Shepherd, J. (2019). Deep learning networks find unique mammographic differences in previous negative mammograms between interval and screen-detected cancers: a case-case study. Cancer Imaging: The Official Publication of the International Cancer Imaging Society, 19(1), 41.
Hirko, K. A., Rocque, G., Reasor, E., Taye, A., Daly, A., Cutress, R. I., Copson, E. R., Lee, D.-W., Lee, K.-H., Im, S.-A., & Park, Y. H. (2022). The impact of race and ethnicity in breast cancer-disparities and implications for precision oncology.BMC Medicine, 20(1), 72.
Hong, R., & Xu, B. (2022). Breast cancer: an up-to-date review and future perspectives. Cancer Communications, 42(10), 913–936. https://doi.org/10.1002/cac2.12358
Hubbard, R. A., Kerlikowske, K., Flowers, C. I., Yankaskas, B. C., Zhu, W., & Miglioretti, D. L. (2011). Cumulative probability of false-positive recall or biopsy recommendation after 10 years of screening mammography: a cohort study.Annals of Internal Medicine, 155(8), 481–492.
Hu, K., Ding, P., Wu, Y., Tian, W., Pan, T., & Zhang, S. (2019). Global patterns and trends in the breast cancer incidence and mortality according to sociodemographic indices: an observational study based on the global burden of diseases. BMJ Open, 9(10), e028461.
Ikeda, D. M. (Ed.). (2011a). Chapter 2 - Mammogram Interpretation. In Breast Imaging (Second Edition) (pp. 24–62). Mosby.
Ikeda, D. M. (Ed.). (2011b). Chapter 5 - Breast Ultrasound. In Breast Imaging (Second Edition) (pp. 149–193). Mosby.
James, J. J., Giannotti, E., & Chen, Y. (2018). Evaluation of a computer-aided detection (CAD)-enhanced 2D synthetic mammogram: comparison with standard synthetic 2D mammograms and conventional 2D digital mammography. Clinical Radiology, 73(10), 886–892.
Kalager, M., Zelen, M., Langmark, F., & Adami, H.-O. (2010). Effect of screening mammography on breast-cancer mortality in Norway. The New England Journal of Medicine, 363(13), 1203–1210. https://doi.org/10.1056/NEJMoa1000727
Kim, Y.-S., Park, H.-S., Lee, H.-H., Choi, Y.-W., Choi, J.-G., Kim, H. H., & Kim, H.-J. (2016). Comparison study of reconstruction algorithms for prototype digital breast tomosynthesis using various breast phantoms. La Radiologia Medica, 121(2), 81–92.
Koo, M. M., von Wagner, C., Abel, G. A., McPhail, S., Rubin, G. P., & Lyratzopoulos, G. (2017). Typical and atypical presenting symptoms of breast cancer and their associations with diagnostic intervals: Evidence from a national audit of cancer diagnosis. Cancer Epidemiology, 48, 140–146. https://doi.org/10.1016/j.canep.2017.04.010
Lång, K., Josefsson, V., Larsson, A.-M., Larsson, S., Högberg, C., Sartor, H., Hofvind, S., Andersson, I., & Rosso, A. (2023). Artificial intelligence-supported screen reading versus standard double reading in the Mammography Screening with Artificial Intelligence trial (MASAI): a clinical safety analysis of a randomised, controlled, non-inferiority, single-blinded, screening accuracy study. The Lancet Oncology, 24(8), 936–944.
Lauritzen, A. D., Rodríguez-Ruiz, A., von Euler-Chelpin, M. C., Lynge, E., Vejborg, I., Nielsen, M., Karssemeijer, N., & Lillholm, M. (2022). An Artificial Intelligence-based Mammography Screening Protocol for Breast Cancer: Outcome and Radiologist Workload.Radiology, 304(1), 41–49.
Lehman, C. D., Yala, A., Schuster, T., Dontchos, B., Bahl, M., Swanson, K., & Barzilay, R. (2019). Mammographic Breast Density Assessment Using Deep Learning: Clinical Implementation. Radiology, 290(1), 52–58.
Leibig, C., Brehmer, M., Bunk, S., Byng, D., Pinker, K., & Umutlu, L. (2022). Combining the strengths of radiologists and AI for breast cancer screening: a retrospective analysis. The Lancet. Digital Health, 4(7), e507–e519.
Lei, J., Yang, P., Zhang, L., Wang, Y., & Yang, K. (2014). Diagnostic accuracy of digital breast tomosynthesis versus digital mammography for benign and malignant lesions in breasts: a meta-analysis. European Radiology, 24(3), 595–602.
Lekadir, K., Osuala, R., Gallin, C., Lazrak, N., Kushibar, K., Tsakou, G., Aussó, S., Alberich, L. C., Marias, K., Tsiknakis, M., Colantonio, S., Papanikolaou, N., Salahuddin, Z., Woodruff, H. C., Lambin, P., & Martí-Bonmatí, L. (2021). FUTURE-AI: Guiding Principles and Consensus Recommendations for Trustworthy Artificial Intelligence in Medical Imaging. In arXiv [cs.CV].arXiv. https://arxiv.org/abs/2109.09658
Liu, J., Zarshenas, A., Qadir, A., Wei, Z., Yang, L., Fajardo, L., & Suzuki, K. (2018). Radiation dose reduction in digital breast tomosynthesis (DBT) by means of deep-learning-based supervised image processing. Medical Imaging 2018: Image Processing, 10574, 89–97.
Liu, X., Cruz Rivera, S., Moher, D., Calvert, M. J., Denniston, A. K., & SPIRIT-AI and CONSORT-AI Working Group. (2020). Reporting guidelines for clinical trial reports for interventions involving artificial intelligence: the CONSORT-AI extension. Nature Medicine, 26(9), 1364–1374.
Łukasiewicz, S., Czeczelewski, M., Forma, A., Baj, J., Sitarz, R., & Stanisławek, A. (2021). Breast Cancer-Epidemiology, Risk Factors, Classification, Prognostic Markers, and Current Treatment Strategies-An Updated Review. Cancers, 13(17). https://doi.org/10.3390/cancers13174287
Lynge, E., Vejborg, I., Andersen, Z., von Euler-Chelpin, M., & Napolitano, G. (2019). Mammographic Density and Screening Sensitivity, Breast Cancer Incidence and Associated Risk Factors in Danish Breast Cancer Screening. Journal of Clinical Medicine Research, 8(11). https://doi.org/10.3390/jcm8112021
Mann, R. M., Cho, N., & Moy, L. (2019). Breast MRI: State of the Art. Radiology, 292(3), 520–536.
Martini, R., Newman, L., & Davis, M. (2022). Breast cancer disparities in outcomes; unmasking biological determinants associated with racial and genetic diversity. Clinical & Experimental Metastasis, 39(1), 7–14.
Mascara, M., & Constantinou, C. (2021). Global Perceptions of Women on Breast Cancer and Barriers to Screening. Current Oncology Reports, 23(7), 74.
McDonald, E. S., Clark, A. S., Tchou, J., Zhang, P., & Freedman, G. M. (2016). Clinical Diagnosis and Management of Breast Cancer. Journal of Nuclear Medicine: Official Publication, Society of Nuclear Medicine, 57 Suppl 1, 9S – 16S. https://doi.org/10.2967/jnumed.115.157834
McKinney, S. M., Sieniek, M., Godbole, V., Godwin, J., Antropova, N., Ashrafian, H., Back, T., Chesus, M., Corrado, G. S., Darzi, A., Etemadi, M., Garcia-Vicente, F., Gilbert, F. J., Halling-Brown, M., Hassabis, D., Jansen, S., Karthikesalingam, A., Kelly, C. J., King, D., … Shetty, S. (2020). International evaluation of an AI system for breast cancer screening. Nature, 577(7788), 89–94.
Mohamed, A. A., Berg, W. A., Peng, H., Luo, Y., Jankowitz, R. C., & Wu, S. (2018). A deep learning method for classifying mammographic breast density categories. Medical Physics, 45(1), 314–321.
Moran, S., & Warren-Forward, H. (2012). The Australian BreastScreen workforce: a snapshot. The Radiographer, 59(1), 26–30.
Nelson, H. D., Fu, R., Cantor, A., Pappas, M., Daeges, M., & Humphrey, L. (2016). Effectiveness of Breast Cancer Screening: Systematic Review and Meta-analysis to Update the 2009 U.S. Preventive Services Task Force Recommendation. Annals of Internal Medicine, 164(4), 244–255.
Nelson, H. D., Pappas, M., Cantor, A., Griffin, J., Daeges, M., & Humphrey, L. (2016). Harms of Breast Cancer Screening: Systematic Review to Update the 2009 U.S. Preventive Services Task Force Recommendation. Annals of Internal Medicine, 164(4), 256–267.
Nguyen, H. T., Nguyen, H. Q., Pham, H. H., Lam, K., Le, L. T., Dao, M., & Vu, V. (2023). VinDr-Mammo: A large-scale benchmark dataset for computer-aided diagnosis in full-field digital mammography. Scientific Data, 10(1), 277.
Ong, M.-S., & Mandl, K. D. (2015). National expenditure for false-positive mammograms and breast cancer overdiagnoses estimated at $4 billion a year. Health Affairs , 34(4), 576–583.
Prevedello, L. M., Halabi, S. S., Shih, G., Wu, C. C., Kohli, M. D., Chokshi, F. H., Erickson, B. J., Kalpathy-Cramer, J., Andriole, K. P., & Flanders, A. E. (2019). Challenges Related to Artificial Intelligence Research in Medical Imaging and the Importance of Image Analysis Competitions. Radiology. Artificial Intelligence, 1(1), e180031. https://doi.org/10.1148/ ryai.2019180031
Raya-Povedano, J. L., Romero-Martín, S., Elías-Cabot, E., Gubern-Mérida, A., Rodríguez-Ruiz, A., & Álvarez-Benito, M. (2021). AI-based Strategies to Reduce Workload in Breast Cancer Screening with Mammography and Tomosynthesis: A Retrospective Evaluation. Radiology, 300(1), 57–65.
Rimmer, A. (2017). Radiologist shortage leaves patient care at risk, warns royal college. BMJ, 359, j4683.
Skaane, P., Bandos, A. I., Niklason, L. T., Sebuødegård, S., Østerås, B. H., Gullien, R., Gur, D., & Hofvind, S. (2019). Digital Mammography versus Digital Mammography Plus Tomosynthesis in Breast Cancer Screening: The Oslo Tomosynthesis Screening Trial. Radiology, 291(1), 23–30.
Sung H et al,(2021). CA Cancer J Clin, 2021 May;71(3):209-249. doi: 10.3322/caac.21660
Tabár, L., Dean, P. B., Chen, T. H.-H., Yen, A. M.-F., Chen, S. L.- S., Fann, J. C.-Y., Chiu, S. Y.-H., Ku, M. M.-S., Wu, W. Y.-Y., Hsu, C.-Y., Chen, Y.-C., Beckmann, K., Smith, R. A., & Duffy, S. W. (2019). The incidence of fatal breast cancer measures the increased effectiveness of therapy in women participating in mammography screening. Cancer, 125(4), 515–523.
Svahn, T. M., Houssami, N., Sechopoulos, I., & Mattsson, S. (2015). Review of radiation dose estimates in digital breast tomosynthesis relative to those in two-view full-field digital mammography. Breast, 24(2), 93–99. https://doi.org/10.1016/j. breast.2014.12.002
Tirada, N., Li, G., Dreizin, D., Robinson, L., Khorjekar, G., Dromi, S., & Ernst, T. (2019). Digital Breast Tomosynthesis: Physics, Artifacts, and Quality Control Considerations. Radiographics: A Review Publication of the Radiological Society of North America, Inc, 39(2), 413–426.
Torres-Mejía, G., De Stavola, B., Allen, D. S., Pérez-Gavilán, J. J., Ferreira, J. M., Fentiman, I. S., & Dos Santos Silva, I. (2005). Mammographic features and subsequent risk of breast cancer: a comparison of qualitative and quantitative evaluations in the Guernsey prospective studies. Cancer Epidemiology, Biomarkers & Prevention: A Publication of the American Association for Cancer Research, Cosponsored by the American Society of Preventive Oncology, 14(5), 1052–1059.
Volpara Health. (2022). TruPGMI: AI for mammography quality improvement. https://www.volparahealth.com/breast-healthsoftware/ products/analytics/
Wing, P., & Langelier, M. H. (2009). Workforce shortages in breast imaging: impact on mammography utilization. AJR. American Journal of Roentgenology, 192(2), 370–378.
Yala, A., Lehman, C., Schuster, T., Portnoi, T., & Barzilay, R. (2019). A Deep Learning Mammography-based Model for Improved Breast Cancer Risk Prediction. Radiology, 292(1), 60–66.
Yala, A., Schuster, T., Miles, R., Barzilay, R., & Lehman, C. (2019). A Deep Learning Model to Triage Screening Mammograms: A Simulation Study. Radiology, 293(1), 38–46.